Salt water ingress in coastal wastewater catchments
By T Miranda, T Hill, R Lockett, M Schnelle and H Bustamante.
First published in Water e-Journal Vol 4 No 2 2019.
Abstract
Salt water ingress (SWI) has become a major issue for coastal wastewater treatment plants due to rising sea levels and ageing infrastructure. Many wastewater assets lie in the tidal zone, allowing salt water to enter wastewater systems through defects. This leads to increased hydraulic load into the system, increased sulfide generation and corrosion of assets, and reduced potential for reuse of treated wastewater.
This paper outlines a successful method used by Sydney Water to measure and locate the sources of SWI into a coastal wastewater catchment, and subsequently target these assets for repair. Minimising SWI reduces operating costs, increases network capacity, and increases the longevity of infrastructure. It is vital to address ingress at the infiltration point for the benefit of both the water utility and its customers.
Introduction
Salt water ingress (SWI) is a widespread and common occurrence affecting coastal wastewater treatment plants (WWTPs) operated by water utilities. When WWTPs and associated assets are near the coast, salt water can potentially enter the system and impact the normal operation of the wastewater network and downstream WWTP. As parts of the coastal wastewater infrastructure are in the intertidal zone, these structures can become inundated during high tides. Salt water can then enter the network through a variety of pathways, including:
- faults and cracks
- backflow into the main wastewater system through emergency relief structures (ERS) (see Figure 1)
- damaged or faulty private house service lines.
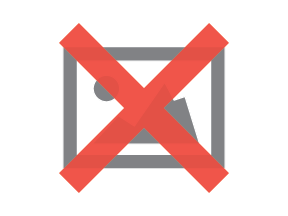
Figure 1 shows how ERS operate. ERS are designed to direct wastewater overflows during high flow events (such as storms) to predetermined locations, such as stormwater systems or waterways. This prevents overloaded sewers from overflowing into public and private properties and impacting customers.
However, under high tide conditions, the level of the receiving water at ERS can rise to a level high enough to backflow through ERS due to faulty one-way valves. This results in salt water ingress into the wastewater networks. The volume of SWI therefore depends on tidal height.
SWI causes issues for both sewage pumping stations (SPS), which retain and pump wastewater through the network, as well as the downstream WWTP. High volumes of salt water entering the network during high tide events can put extra hydraulic load on SPS with limited capacity, potentially causing wastewater overflows to the environment. As part of Sydney Water’s regulatory licensing, SPS overflows are not permitted. In addition, correlation between H2S gas generation and tidal ingress (increase of around 8 ppm on a high tide day) will impact rates of asset corrosion and increase odour risk. SWI increases flows and reduces spare capacity in the network and volumes of wastewater required for treatment at WWTPs. This can potentially necessitate expensive capacity upgrades.
Cronulla Wastewater Treatment Plant (WWTP) services a residential catchment in Sydney’s south, with average dry weather flow of 53 ML/day serving a population of approximately 250,000 people. An unknown change in the network caused a spike in wastewater effluent salinity levels in May 2017, warranting an investigation to identify and target sources of SWI within its catchment. Additionally, Cronulla WWTP sends approximately 4 - 6 ML/day of its final effluent to a downstream water recycling plant (WRP) as part of a community sewer mining initiative for irrigation. Generally, conductivity values above 3,000 µS/cm are considered ‘severe’ and impact the suitability of water for irrigation (Metcalf & Eddy 2003). When affected by saline effluent, the downstream WRP dilutes the recycled water with potable water to reduce the impact of the salinity on irrigation. Considering the added impetus of drought and water restrictions, eliminating the need to dilute with potable water is beneficial for all stakeholders.
Normal operating conditions for Cronulla WWTP included average conductivity readings of approximately 1,500 µS/cm. The shift in the Cronulla network from May 2017 led to a step change increase in conductivity above the baseline, with readings exceeding 5,000 µS/cm during high tide (> 1.7 m) and king tide (> 2 m) events.
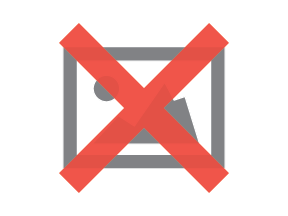
The purpose of this investigation was to understand the increase in salinity at Cronulla WWTP from May 2017 and target areas for repair. To do this, we analysed flow and salinity at the WWTP, the three major submains (Figure 2), and their associated SPS catchments during significant tidal events. Concurrently, we continuously measured conductivity of the WWTP effluent to monitor salinity levels and validate the progress of repairs on the network.
The key questions addressed in this paper are:
- What is the extent of SWI into the Cronulla network?
- Can we identify and target the impacted areas of the network?
- How successful were the repairs in reducing SWI?
Method
1. Measuring and locating sources of SWI
The investigation was conducted in three spatial stages, beginning from the downstream WWTP effluent, working upstream to submains then to various SPS to delineate the key sources of SWI into the Cronulla system.
a) At Cronulla WWTP
Conductivity (µS/cm) was used as an indicator of salt water concentration as it is a source of charged ions. Conductivity data was sourced from a continuous online conductivity meter at the outlet of Cronulla WWTP, which measures conductivity levels at one-minute intervals. Effluent conductivity was measured due to potential biofouling and blockage issues at the influent point. As no salt is removed or added during the treatment process, conductivity readings at the influent and effluent were assumed to be equivalent. Initially, to understand the extent to which salinity increased at Cronulla WWTP, historic conductivity grab sample data from 2005–2017 was analysed.
Comparison of coastal and inland WWTPs
Conductivity was compared between several inland and coastal WWTPs to determine the impact of SWI on measured salinity at coastal treatment plants. This was sourced from historic WWTP data from 2005 to 2017 with a sampling frequency of six days. A one-way Analysis of Variance (ANOVA) was performed to determine if there was a statistically significant difference between coastal and inland WWTPs.
Tidal analysis
To explore the relationship between conductivity and tides, we compared conductivity over a neap (low) tide event (1.1 m on 27 December 2017) and king tide event (2.24 m on 3 January 2018). These days were on an equivalent day exactly one week apart, with equivalent rainfall conditions (negligible rainfall).
It was noted that as the neap and high tide comparison was performed over a holiday period (27/12/17 – 03/01/18), data may not be representative of typical water use. However, as both events occurred during the holiday period, a meaningful comparison could still be made.
A one-way ANOVA was performed to determine if there was a statistically significant difference in conductivity between these two events.
Predictive model
The correlation between various conductivity and tide parameters was assessed using the R statistical analysis software function cor. As the correlation between maximum conductivity and maximum tide was the highest, a model was developed to predict salinity ranges at the WWTP based on forecasted tide heights. The model allowed a range of tide heights to be input and using the best predictive fit for current data (a second order polynomial linear regression), a typical range of expected conductivity was output.
b) Within network submains
Once we established a correlation between salinity and tides at the WWTP, we began to delineate and locate the sources of SWI within the wastewater network feeding to the WWTP. These areas could then be prioritised for repairs. We began by moving upstream to the major submains in the Cronulla network that fed into the WWTP (Figure 2), using existing flow gauge data. All flow data was sourced using Sydney Water’s Integrated Instrumentation Control Automation and Telemetry System (IICATS).
Flow rates at selected locations along the submains were compared over the neap tide event (1.1 m) and king tide event (2.24 m). Similarly, the inlet flow rate (in L/s) to Cronulla WWTP was compared over the same neap tide and king tide events to confirm the patterns observed in the network. A two-way ANOVA was conducted to determine if there was a statistically significant difference between the two tide events on flow rates, by controlling for the effect of flow gauge location.
c) Within SPS catchments
Once we established which submains were contributing to SWI, we moved further upstream along those submains to target specific SPS. We identified and assessed ten salt water impacted SPS and analysed flow volumes (ML/day) during four separate tidal events (Table 1).
An assumption was made that any extra flow observed at the SPS during tidal events could be attributed to salt water. By calculating the extra flow and dividing by the total flow, we could determine the percentage of salt water in the total flows from these ten affected SPS during each event.
Additionally, the contributions from each affected SPS on the king tide event was determined by the following calculation:
This estimated the severity of SWI at each individual SPS and delineated the major sources of ingress into the Cronulla system. Repair works could then be prioritised to maximise value.
Virtual inflow analysis
While there are several existing flow gauges in submains throughout the Cronulla network, there are no flow meters in SPS. For this reason, in addition to determining the increase in pump running hours, an IICATS virtual inflow meter tool was used to analyse the effect of tide height at SWI impacted SPS. The virtual inflow meter estimates inflows based on the change in level of the SPS over time. This was used to determine tidal infiltration, indicative inflow rates and volumes to SPS wells during tidal events. The correlation between tide and inflow rate was determined using the R function cor.
2. Repairing sources of SWI
Once sources of SWI were delineated to specific SPS catchments, a targeted investigation program was carried out using tools such as CCTV and SewCam (Hill & Hearfield 2018) to visualise the extent of SWI. When faults were identified, a program of repairs and maintenance was initiated. Works included repairing manhole faults, repairing faulty private house connections, and replacing ERS valves.
3. Validation analyses
To validate the success or failure of the targeted repairs, the mid-year king tides were used as a comparison. These king tides peaked at 2.11 m on 18 June 2018.
Average conductivity for a range of tide levels was compared between the months of January 2018 and June 2018. Values for January were used to reflect the network pre-repairs, and values for June were used to indicate progress after repairs had commenced.
As the January 2018 king tide peaked at 2.24 m and the June 2018 king tide peaked at 2.11 m, conductivity was compared up to the 2.11 m tide level in order to make a meaningful comparison.
An Analysis of Covariance (ANCOVA) was conducted to determine a statistically significant difference in conductivity between both the variables tide height and month.
All statistical analyses were performed in R version 1.1.442 (R Core Team 2013).
Results and discussion
1. Measuring and locating sources of SWI
a) At Cronulla WWTP
Analysis of historical data indicated that Cronulla WWTP had an upward trend in conductivity measurements in 2017. Mean conductivity for Cronulla historically has been 1,482.48 ± 332 µS/cm (Figure 3).
Mean conductivity values for Cronulla are slightly higher than other values from the literature which are generally within the range of 500-1,000 µS/cm (Odjadjare & Okoh, 2010; Levlin 2010). This suggests that Cronulla may have had historical baseline ingress issues. Due to the difficulty in determining baseline SWI sources, these were classified as out of scope for this particular investigation.
Comparison of coastal and inland WWTPs
Historically, conductivity has been relatively low at inland plants, well below 1,500 µS/cm, despite factors such as the unknown impact of seasonal fertiliser application by customers in some inland wastewater networks. In comparison, Cronulla WWTP has often surpassed 2,000 µS/cm and has experienced peaks up to 6,000 µS/cm. Statistical analyses showed that there was an overall difference in conductivity between inland and coastal plants (ANOVA, F(1,1900) = 559.1, P<0.001; Figure 4).
Tidal analysis
Analysis of current conductivity data from Cronulla WWTP’s continuous monitor showed a clear correlation between effluent conductivity and tidal height, with a difference of >3,550 µS/cm between a high (king tide) and low (neap tide) event (Figure 5). Statistical analysis also indicated a significant difference in conductivity between the two events (ANOVA, F(1, 547) = 423,538, P<0.001).
Given that baseline (historical) average conductivity levels at the WWTP are approximately 1,500µS/cm, online conductivity readings measured from August 2017 – around 1,800µS/cm on average – indicated a salinity increase of approximately 20%.
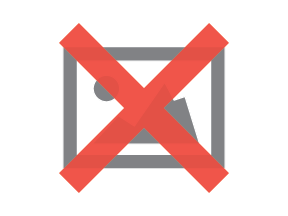
Predictive model
The correlation value between maximum tide height and maximum conductivity at the WWTP was 0.74, indicating a strong relationship between the two parameters (Figure 6).
The model developed from these variables could predict an indicative range of conductivity in response to forecasted tide heights. For example, if a tide height of 1.8 m was forecast and we wanted to understand the range of conductivity at the WWTP following that tide event, the model produced a conductivity range between 1,981 and 2,370 µS/cm (Figure 7).
This tool provided an interim early-warning system to take place between Cronulla WWTP and affected stakeholders while the investigation was conducted. This method can also potentially be replicated to predict salinity ranges in other coastal catchments.
b) Within network submains
Results indicated that there was a significant effect of tide on the flow rates along the submains (Two-way ANOVA, F(1,3449) = 16.05, P<0.001; Figure 8). The flow gauge analysis demonstrated that flows increased in submain catchments with inundated assets during tidal events.
The greatest change in wastewater flow was observed to be an increase of 36 L/s (Figure 8), within the northern Sutherland submain.
Analysis of the Cronulla WWTP inlet flow also showed that SWI was impacting the average inlet flow rate, with a peak increase of 61 L/s observed over the two events.
C) Within SPS catchments
As much of the impact was observed in SPS within the Sutherland and Cronulla submains, these were analysed to identify the major contributors to SWI. Ten SPS were identified to be ‘at risk’ due to their location within the area of tidal inundation. Calculated data showed that increased flows through affected SPS increased with tide height (Figure 9).
The results in Table 2 indicate that on the 2.24 m king tide event on 3 January 2018, flows from these tidally affected SPS increased by 140% above baseline conditions, which could be indicative of salt water ingress.
As the total influent volume at Cronulla WWTP averages around 47.73 ML/day, we estimated that the SWI-attributed flows from the ten affected SPS accounted for approximately 4% of total inflow to the plant on the king tide day. As the inflow occurs over high tide period only, and only in a few affected SPS, this inflow has a localised high impact well above the 4% average amount for the catchment across the whole day. Higher risk of the system exceeding capacity would occur if tidal events align with existing diurnal flow peaks or heavy rainfall observed in the catchment.
The following SPS were found to be the major contributors of SWI in the Cronulla catchment: Sylvania (23%), Kangaroo Point (14%), Woronora (12%) and Taren Point (11%).
Virtual inflow analysis
Analysis using the Virtual Inflow Analysis tool indicated a strong correlation between tides and inflow to SPS (Figure 10), with the highest correlation between tide and inflow rate (correlation factor = 0.63). This supports the findings from the previous flow analyses conducted on the submains.
Inflow data also demonstrated that infiltration to SPS occurs at tides above 1.62 m based on a 95% lower confidence interval of the mean infiltration height (1.74 m ± 0.124). Therefore 1.62 m was determined as the threshold after which higher tide levels begin to substantially impact the Cronulla system.
2. Repairing sources of SWI
Once the key sources of SWI were delineated to the Sylvania, Kangaroo Point, Woronora and Taren Point SPS catchments, a targeted repair and maintenance program of works was initiated to reduce SWI into the system. Repairs were conducted between February and May 2018, and included replacing maintenance hole lids, repairing cracks and faults, repairing damaged connections and installing backflow prevention valves in ERS. As most infiltration in the system occurred at tide heights greater than 1.62 m, we focussed the repair effort on assets located at that height or greater.
Visual observations in the Kangaroo Point SPS catchment uncovered a number of damaged private house lines, with some salt water entering the system during tides as low as 1.3 m.
In-situ conductivity monitoring in a maintenance hole on a 1.5 m high tide day showed that almost 50% of water in the main network was salt water, with conductivity readings at approximately 25,000 µS/cm. Additionally, it was observed that a particular ERS began to contribute salt water into the main sewer network at tides of approximately 1.7 m and above, due to backflow through a faulty valve.
Visual monitoring of the system using CCTV analysis estimated that one third of the ingress to the Cronulla system was attributed to private house lines, one third to damaged maintenance holes, and one third to ERS backflow (Hill & Hearfield, 2018).
3. Validation analyses
Analysis of conductivity between the January 2018 and June 2018 king tides showed that there was a significant difference in conductivity between both tide height (ANCOVA, F(7,52742) = 413.9, P<0.001) and month (ANCOVA, F(7,10) = 735.7, P<0.001).
Results therefore indicate that conductivity levels before and after repairs significantly differ (also indicated by the distinctiveness of standard error bars in Figure 11). This suggests repairs in targeted areas of the catchment have significantly reduced overall SWI and thus salinity levels in the system.
Conductivity levels between the January 2018 and June 2018 king tide events decreased by 39%, demonstrating the success of repair efforts so far. Outstanding repair efforts in the catchment are expected to reduce this further.
Reducing salt water ingress will decrease the volume of wastewater to be treated, reduce the impact of corrosion, and enable Sydney Water to maintain the provision of effluent quality used in the downstream community recycled water scheme. SWI reduction efforts have reduced the risk of SPS overflows during high tide events, protecting the environment, and allowing Sydney Water to continue to meet its licence requirements. The SWI reduction initiative will also provide substantial cost savings due to reduced pump run times.
Conclusion
This study quantified the extent of SWI in the Cronulla wastewater system. This enabled the identification of major sources of ingress and targeted repair efforts. Flow gauge and SPS data confirmed that SWI increased with tide height, and virtual inflow data confirmed that substantial ingress occurred from tides above 1.62 m.
The study developed a methodology to identify and repair sources of SWI. The methodology involved developing a model to predict increases in conductivity associated with tides and provide an interim early-warning system for affected stakeholders. The model can also be replicated to quantify SWI across other coastal catchments.
Using the methodology, it was possible to identify the most affected areas in the catchment. Repair works were then prioritised to high impact sites to maximise value.
The average conductivity was compared for the two major 2018 king tide events, with an observed reduction of 39% after the first round of repair works. Ongoing online conductivity monitoring allowed us to track progress and validate the success of the repairs conducted, including identifying any future increase in SWI from newly damaged assets.
This study successfully demonstrated an approach to mitigating the issue of SWI in coastal wastewater systems. Learnings from this case study will strengthen Sydney Water’s SWI strategy so that an optimised process and framework for SWI reduction can be applied to other affected wastewater catchments across the Sydney region.
Acknowledgements
A special thank you to all those who contributed to this case study, including Robert Aurisch (Cronulla WWTP Plant Manager) and David Cantlon (Sydney Water Networks South Manager) who endorsed and supported the trial. Additional thanks to contributors and reviewers, including Mark McGowan, Frank Vidovic and Elliot Cichero.
About the authors
Tashya Miranda | Tashya Miranda graduated with an Honours degree in Environmental Science from the University of New South Wales. She spent a year working as an Environmental Consultant specialising in Contaminated Land Management. She then moved to Sydney Water, where she joined the Science, Research and Innovation team and contributed to large scale research projects. She now works in Sydney Water's operations division, conducting water quality analysis, data analysis and reporting.
Heri Bustamante | Dr Heriberto (Heri) Bustamante is a Principal Research Scientist, Treatment at Sydney Water. One of his roles is to identify research needs in Sydney Water’s operations and convert them into research projects to be carried out in collaboration with local and international universities. Heri is currently working on collaborative projects with UNSW (graphene oxide membranes for NOM rejection), Macquarie University (Photonic sensors to manage sewer corrosion and ventilation) and with UTS on co-digestion and downstream effect (causes of high polymer demand in sludge conditioning)
Matthew Schnelle
Matthew Schnelle graduated with an honours degree in Chemical Engineering from the University of Sydney. Since joining Sydney Water in 2017, Matt has worked in product quality, service planning and process optimisation, including a year at Cronulla Wastewater Treatment Plant. Matt now works in Sydney Water’s operations team as a planner for water and wastewater networks.
Rebecca Lockett | Rebecca Lockett graduated with an Honours degree in Chemical Engineering from the University of Sydney. She spent her first year at Sydney Water working at Bondi Wastewater Treatment Plant. She then transitioned into corrosion and odour management in wastewater networks. Rebecca is now working in a consulting team for potable water filtration plants.
Timothy Hill | Timothy Hill has a degree in Environmental Science from Australian Catholic University. He joined Sydney Water in 2006 and has worked in analytical and field services for ten years. His focus has been around monitoring of environmental and waste waters in an assessment and reporting capacity. Timothy joined Sydney Water network services in 2016 working in programs, operations and regulatory reporting around the management of environmental incidents, pressure mains, salt water ingress and silt. He has been focussed on innovative methods of monitoring including development of drones for water sampling and video-graphic monitoring methods.